Editor: Nina
Scientists develop a shortwave-infrared light-emitting nanoparticle-based nanovaccine to enable real-time tracking of immune responses and effective eradication of cancer tumors in vivo.
Key Preview
Research Question
This study investigates how shortwave-infrared (SWIR) light-emitting probes can be utilized for the in vivo tracking of cancer vaccines and the immune responses they trigger.
Research Design and Strategy
The research employs a novel approach that combines the development of a cancer vaccine with imaging technology to monitor immune responses in real-time. The study focuses on using polymer-coated nanoparticles emitting in the near-infrared spectral window for improved imaging depth and resolution.
Method
The researchers synthesized polymer-coated NaErF4/NaYF4 core-shell down-conversion nanoparticles and conjugated them with antigens and adjuvants. The vaccine was administered subcutaneously to tumor-bearing mice, and the migration of nanoparticles was tracked using wide-field and lifetime imaging techniques.
Key Results
The study found that two doses of the vaccine led to the complete eradication of pre-existing tumors in mice, demonstrating a strong correlation between vaccine efficacy and the abundance of antigen-specific CD8+ T lymphocytes in the tumor microenvironment.
Significance of the Research
This research signifies a critical advance in cancer immunotherapy, providing a dual-function tool that enhances our understanding of vaccine efficacy and immune response while enabling non-invasive in vivo monitoring.
Introduction
Cancer remains one of the leading causes of morbidity and mortality worldwide, characterized by the uncontrolled proliferation of abnormal cells. The complexity of cancer biology, including its ability to evade the immune system, presents significant challenges in treatment. Traditional cancer therapies, such as chemotherapy and radiotherapy, often employ non-specific drug delivery methods, where cytotoxic agents are administered systemically. This approach can lead to a range of adverse effects due to the indiscriminate targeting of both cancerous and healthy cells, resulting in significant toxicity and limiting the overall effectiveness of the treatment.
Despite advancements in cancer treatment, the conventional strategies often fail to achieve desired therapeutic outcomes due to several key challenges. One major issue is the limited specificity of drugs, which can result in suboptimal concentrations reaching the tumor site while healthy tissues suffer from the toxic effects of treatment. Additionally, the presence of a dense tumor stroma and aberrant vasculature can impede drug penetration, further diminishing the efficacy of systemic therapies. These challenges contribute to treatment resistance, disease recurrence, and ultimately, reduced survival rates among cancer patients.
To overcome these limitations, innovative drug delivery strategies are being explored. One promising approach involves the use of nanoparticles, which can be engineered to enhance drug delivery specifically to tumor sites. These nanoparticles can be designed to improve drug solubility, protect therapeutic agents from degradation, and facilitate selective uptake by cancer cells. In particular, the integration of imaging capabilities within the nanoparticles allows for real-time monitoring of drug delivery and distribution within the body, providing critical insights into the therapeutic response. This study focuses on the development of shortwave-infrared light-emitting probes that can track cancer vaccines and the immune responses they elicit, aiming to optimize cancer immunotherapy through enhanced drug delivery and monitoring.
Research Team and Aim
The research was conducted by a collaborative team from Stanford University, led by Dr. Hongjie Dai, a prominent figure in the field of nanotechnology and its applications in biomedical research. This study was conducted between 2022 and 2023, culminating in the publication of the paper titled “Shortwave-infrared-light-emitting probes for the in vivo tracking of cancer vaccines and the elicited immune responses” in the journal Nature Biomedical Engineering.
The aim of the research, as articulated by Dr. Dai, was to “design a nanovaccine that could be tracked in real-time, thereby providing insights into the mechanisms of vaccine-induced immune responses.” This innovative approach not only seeks to advance our understanding of vaccine efficacy but also to enhance the monitoring capabilities of immune responses in cancer therapy.
Experimental Process
Experiment 1: Synthesis of Nanoparticle Probes
Primary Technique:
The primary technique employed in this study was the synthesis of polymer-coated NaErF4/NaYF4 core-shell down-conversion nanoparticles. This method was selected for its efficiency in producing nanoparticles that emit light in the near-infrared spectral window, crucial for deep tissue imaging.
Key Steps:
- Synthesis of Core Nanocrystals: Initiate the synthesis by mixing erbium (III) acetate hydrate, oleic acid, and 1-octadecene in a flask and heat to 150 °C for 30 minutes.
- Addition of Precursors: After cooling, add a methanol solution of ammonium fluoride and sodium hydroxide, stirring for one hour at 50 °C.
- Temperature Increase: Gradually heat the mixture to 300 °C and maintain for 60 minutes, then increase to 305 °C for an additional 19 minutes before cooling to room temperature.
- Washing and Collection: Collect the nanoparticles via centrifugation, wash with ethanol, and disperse in cyclohexane for further coating.
Data Collection and Analysis:
The synthesized nanoparticles were characterized using transmission electron microscopy (TEM) to assess size and morphology, and dynamic light scattering (DLS) was employed to determine the hydrodynamic size and stability in solution.
Figure 1. (a) The histograms of pErNP TEM images show that the size of the nanoparticles is ~ 21.7 nm. (b) Low-resolution TEM images of pErNP. © and (d) High-resolution TEM images of pErNP revealing the core/shell structure and clear lattice fringes with a distance of 0.513 nm corresponding to the d spacing of the (100) lattice planes of the hexagonal structure. The shell thickness is about 3.8 nm. To prepare the pErNP sample for TEM analysis, a 10 mg/ml solution of pErNPs in cyclohexane was dropped onto a 400-mesh copper carbon film. The sample was imaged using a FEI Tecnai G2 F20 X-TWIN Transmission Electron Microscope.
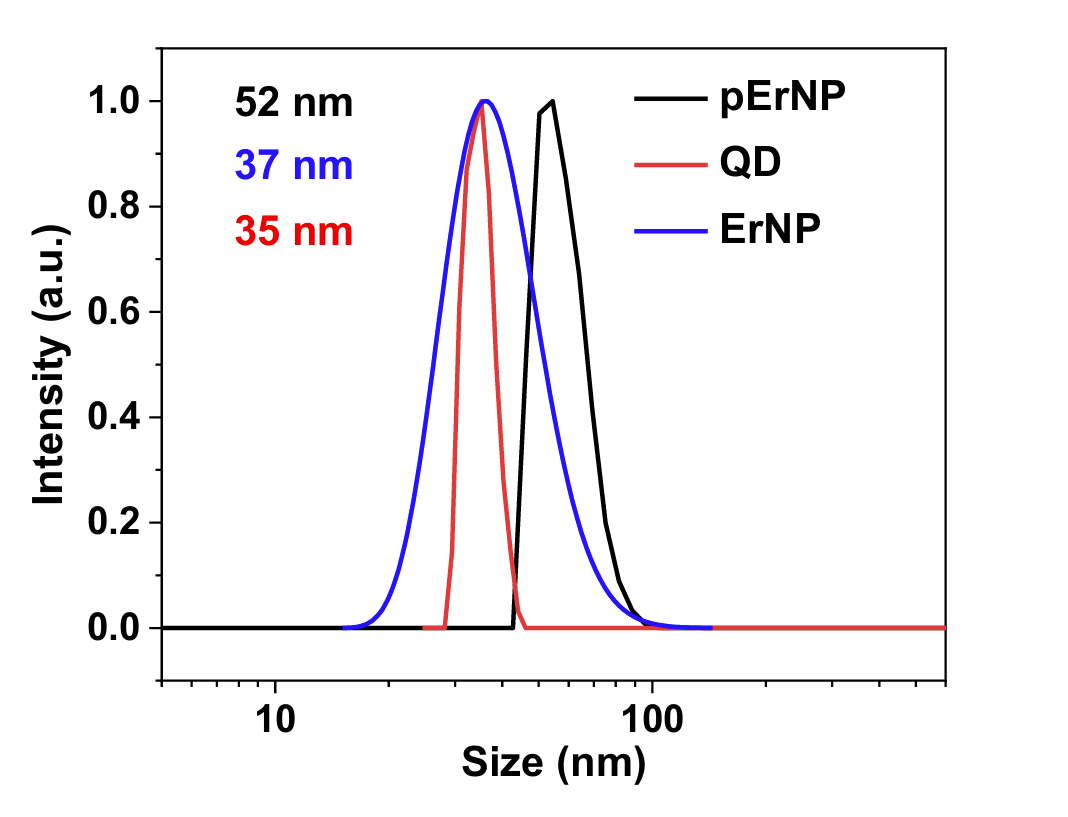
Figure 2. Dynamic light scattering (DLS) spectra of hydrophilic pErNP-P3, QD-P3 and ErNP-P3 measured in PBS buffer. The average hydrated sizes for these three particles are 52 nm, 35 nm, and 37 nm, respectively. The samples were analyzed by DLS in PBS buffer using a Malvern Zetasizer Nano ZS90.
Result:
The synthesized NaErF4/NaYF4 nanoparticles exhibited a uniform size of approximately 21.7 nm, confirming successful synthesis. This size is optimal for lymphatic transport and cellular uptake.
Novel Aspects:
The innovative aspect of this experiment lies in the use of a polymer coating that enhances biocompatibility and facilitates effective conjugation with antigens and adjuvants. This approach significantly improves upon traditional nanoparticle delivery systems by providing a dual function of drug delivery and imaging.
Experiment 2: Vaccine Formulation
Primary Technique:
The secondary experimental technique involved the formulation of the pErNP–OVA–CpG B nanocomplex. This was performed using a conjugation strategy that integrates the nanoparticle with a model antigen and an adjuvant.
Key Steps:
- Conjugation of Antigen: Mix the synthesized pErNPs with ovalbumin (OVA) in a phosphate-buffered saline (PBS) solution, using EDC chemistry to facilitate the reaction.
- Complexation with CpG B: After confirming successful conjugation of OVA (with ~95.6% efficiency), add CpG oligodeoxynucleotide to the mixture, allowing electrostatic interactions to form the final nanocomplex.
- Purification: Remove unbound materials through centrifugation and dialysis to ensure a pure vaccine formulation.
Data Collection and Analysis:
The conjugation efficiency was quantified using fluorescence spectroscopy, and zeta potential measurements were taken to confirm the stability of the nanocomplex in solution.
Result:
The final pErNP–OVA–CpG B formulation demonstrated a successful encapsulation of both OVA and CpG B, with a stable zeta potential of approximately +3.8 mV, indicative of favorable interactions for in vivo applications.
Novel Aspects:
This experiment introduces a sophisticated dual-delivery system that combines imaging and therapeutic properties in a single nanoparticle-based vaccine platform, enhancing the potential for targeted immune responses compared to traditional vaccine formulations.
Experiment 3: In Vivo Tracking of Vaccine Trafficking
Primary Technique:
The primary technique for this experiment was in vivo wide-field NIR-II fluorescence imaging, used to track the biodistribution and trafficking of the pErNP–OVA–CpG B nanovaccine in tumor-bearing mice.
Key Steps:
- Administration of Vaccine: Subcutaneously inject the pErNP–OVA–CpG B formulation at the base of the tail in C57BL/6 mice bearing E.G7-OVA tumors.
- NIR-II Imaging: Perform fluorescence imaging at various time points post-injection (e.g., 0, 6, 12, and 24 hours) using an InGaAs camera with a 975 nm excitation wavelength and a detection range of 1,500–1,700 nm.
- Data Capture: Capture images and record the intensity of the NIR-II signal in the inguinal lymph nodes (iLNs) and at the injection site.
Data Collection and Analysis:
Analysis involved measuring the intensity of fluorescence signals in the iLNs and correlating these with time post-injection to assess the kinetics of vaccine trafficking. Statistical analysis was performed using two-tailed t-tests to determine significance.
Figure 3.The tumour-to-normal tissue (T/NT) signal ratios of ErNP–aCD8 and QD–tetramer in tumour plotted as a function of time. T/NT was measured by ImageJ/Fiji using the ratio of fluorescence signals in the whole tumour area over the background without vasculature. For immunized group: ErNP–aCD8 versus QD–OVA–tetramer, P < 0.0001; QD–RBD–tetramer versus QD–OVA–tetramer, P = 0.0012; for control group: ErNP–aCD8 versus QD–OVA– tetramer, P = 0.4503. All data are from three independent experiments and are presented as mean ± standard deviation. Two-sided Student’s t-tests were used for the comparisons.
Result:
The results indicated a notable increase in fluorescence intensity in the iLNs, peaking at approximately 6 hours post-injection, which correlated with effective vaccine trafficking to lymph nodes essential for immune activation.
Novel Aspects:
The innovative use of NIR-II imaging allows for real-time, non-invasive monitoring of vaccine distribution, providing insights into the kinetics of immune response activation that are not possible with traditional imaging methods.
Experiment 4: Immunological Efficacy Assessment
Primary Technique:
The primary technique for assessing the immunological efficacy of the nanovaccine was flow cytometry, used to quantify antigen-specific CD8+ T cell responses.
Key Steps:
- Tumor Inoculation: E.G7-OVA tumor cells were inoculated into the left hindlimb of mice to establish tumors.
- Vaccination Protocol: Administer the pErNP–OVA–CpG B nanovaccine on specified days, followed by a booster.
- Collection of Tumor Samples: After treatment, dissect tumors, digest them using collagenase and DNase, and prepare single-cell suspensions.
- Flow Cytometry Staining: Stain the cells with OVA-specific SIINFEKL-H-2Kb tetramer and anti-CD8 antibodies, followed by flow cytometry analysis to quantify the percentage of CD8+ T cells responding to the vaccine.
Data Collection and Analysis:
Flow cytometry data were analyzed using FlowJo software to determine the frequency of OVA-specific CTLs in tumor tissue, providing quantitative insights into the immune response.
Figure 4. d, The same as in b (mouse immunized with pErNP– OVA–CpG B nanovaccine) except that QD–RBD–tetramer was used instead of the QD–OVA–tetramer.
Result:
The vaccinated mice showed a significantly higher percentage of OVA-specific CD8+ T cells in the tumor microenvironment compared to control groups, validating the efficacy of the nanovaccine.
Novel Aspects:
This experiment emphasizes the ability of the nanoparticle-based vaccine to elicit a robust, antigen-specific immune response, highlighting its potential as an effective immunotherapeutic strategy in cancer treatment.
Conclusion
The successful development of this innovative drug delivery system was achieved through a combination of advanced material synthesis, precise formulation strategies, and the integration of real-time imaging capabilities. The research team effectively synthesized polymer-coated NaErF4/NaYF4 core-shell nanoparticles that not only serve as a vehicle for delivering cancer vaccines but also emit light in the shortwave-infrared (SWIR) range, facilitating non-invasive tracking of vaccine distribution in vivo. By leveraging the unique properties of these nanoparticles, the team was able to monitor the biodistribution and immune responses triggered by the vaccine in real time.
A highlight of the study is the demonstration that the pErNP–OVA–CpG B nanovaccine achieved complete eradication of pre-existing tumors in tumor-bearing mice, with a remarkable correlation between vaccine efficacy and the abundance of antigen-specific CD8+ T lymphocytes in the tumor microenvironment. This groundbreaking work not only provides critical insights into the mechanisms of vaccine-induced immune responses but also sets the stage for the design and optimization of more effective cancer immunotherapies in the future. By overcoming traditional challenges in drug delivery, this research represents a significant advancement in the field of cancer treatment.
Reference
Ren, Fuqiang, et al. “Shortwave-infrared-light-emitting probes for the in vivo tracking of cancer vaccines and the elicited immune responses.” Nature Biomedical Engineering, vol. 8, no. 6, 2024, pp. 726-739. DOI: 10.1038/s41551-023-01083-5.